Thermodynamics and safety
According to the source2, thermodynamics is arguably the most universal physical theory, primarily due to its wide range of applications; it helps to understand, for example, the functioning of internal combustion engines, the physical properties of condensed matter, and processes occurring in stars and galaxies. Identical thermodynamic laws apply to both classical and quantum systems. The subject of thermodynamics is therefore thermodynamic (macroscopic) systems, i.e., systems that can be uniquely and completely described using a few physical parameters (e.g., density, volume, elasticity, polarization, magnetism, concentration, pressure, etc.).
Thermodynamic systems are classified based on their interaction with the surroundings as:
isolated – do not interact with the surroundings; they have constant energy, volume, and number of particles,
closed – have constant volume and number of particles, but energy exchange with the surroundings occurs,
open – there is exchange of both energy and matter (number of particles).
Based on the uniformity of the system, we further classify systems as homogeneous (with uniform properties throughout the system) or heterogeneous.
Depending on the type of problem being solved, thermodynamics is divided into:
general (or physical) – basic principles,
technical – applications of general thermodynamics for the construction of thermal machines,
chemical – applications in systems with physical, physical-chemical, and chemical processes.
Thermodynamics is based on six postulates,
which were created by generalizing observable and experimentally verifiable facts, source3:
1. Postulate – about the transition of a system to equilibrium state: “Under constant external conditions, every system will reach a state of thermodynamic equilibrium”.
2. Postulate – “Internal energy U is a state extensive quantity”, i.e., U is influenced by: the sum of the kinetic energy of moving particles; potential energy due to mutual attraction and repulsion of particles; radiation energy within the system. That is, U is not influenced by: the motion and position of the system as a whole.
3. Postulate – the so-called 0th law of thermodynamics: “If two distinct bodies A and B are in thermal equilibrium (i.e., they have the same temperature) with body C, then they are in thermal equilibrium (i.e., they have the same temperature) with each other.” – i.e., if Ta = Tb and Ta = Tc => Ta = Tc.
4. Postulate – First law of thermodynamics described below.
5. Postulate – Second law of thermodynamics described below.
6. Postulate – Third law of thermodynamics described below.
Laws of thermodynamics (theorems)
I. law of thermodynamics
The first law of thermodynamics is an expression of the law of conservation of energy in thermodynamics, which describes the relationship between heat, work, and the internal energy of a thermodynamic system. The internal energy of the system (or the system) is, according to4, a definite function of its state and only changes under the influence of external factors.
From the perspective of theoretical physics, the first law of thermodynamics is formulated as follows:
ΔU = W + Q, (1)
From the perspective of technical thermodynamics, it is more advantageous to define the first law of thermodynamics using the equation:
đQ = dU + đW, (2)
where đQ is the heat supplied to the thermodynamic system, which is used to change its internal energy dU and perform work đW of the system. Technical thermodynamics, according to (J. GRUBER)5, considers work đW to be positive if the system is doing work and negative if the system is consuming work; heat đQ is positive if it is supplied, negative if the system releases it.
II. law of thermodynamics
The above-mentioned sources provide the following formulations:
Thomson-Planck – It is not possible to construct a periodically working machine that would cause no changes other than doing work based on removing a constant amount of heat from sources at a constant temperature.
Carnot-Clausius – Heat cannot spontaneously flow from a colder body to a hotter one.
From Carathéodory’s formulation, a mathematical description can be derived: “In every neighborhood of any state of a thermally homogeneous system, there exist states to which it is not possible to approach arbitrarily by an adiabatic change of state parameters.“
The mathematical formula uses the quantity of entropy S, and the change in entropy of an isolated system can be mathematically expressed as the amount of heat transferred per unit temperature:
dS = đQ / T. (3)
Important insights for the construction of heat engines are (see also Figure 1):
– every heat engine must operate between two heat reservoirs,
– it takes thermal energy from the reservoir at a higher temperature,
– part of the energy taken is converted into work,
– the remaining energy is transferred to the reservoir at a lower temperature.
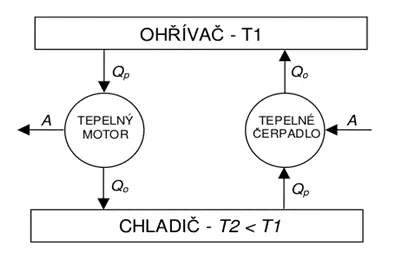
III. law of thermodynamics
Planck – The entropy of a pure phase approaches zero as temperature decreases:
limT→0 S = 0. (4)
A pure solid substance cannot be cooled to absolute zero Kelvin temperature by a finite process[1]. From this, it follows that the absolute value of entropy can be calculated for elements and compounds. Entropy allows for a graphical representation of the amount of heat supplied and removed.
Thermodynamic event
Thermodynamic processes are transitions of systems from one state to another. According to the source 7:
– The values of state variables (e.g., internal energy of the system) do not depend on the way the change occurred; they change regardless of the path taken for the change.
– The values of non-state variables depend on the way the change occurred.
Processes are further divided into: cyclic (circular); reversible and irreversible (reversible / irreversible); and processes at constant thermodynamic variables.
Cyclic action, thermal efficiency
If we consider a periodically (in a cycle) working machine, then the working substance must return to its original state (closed cycle – see figure 2), or a substance with constant initial parameters must be supplied (open cycle – see figure 1 with constant parameters) – i.e., the initial internal energy is equal to the final internal energy of the system. A portion of heat must constantly be discharged, as the system would continuously heat up with an increase in internal energy, because it is practically impossible for all the supplied heat Op to be converted into work A).
The technical work of the cycle At is equal to the absolute work obtained A1 minus the absolute work returned to the cycle A2 (J. GRUBER):
At = A1 – A2. (5)
As stated earlier: “only a portion of the supplied heat (Qp) can be used to do work (At), the remaining portion must be discharged as waste heat (or discharged Qo).” Therefore, the relationship holds:
Qt = At – Qo. (6)
The theoretical measure of the utilization of supplied energy is thermal efficiency (J. GRUBER):
η = (Qp – Qo) / (Qp) = 1 – (T2 / T1), (7)
Since it is practically impossible for the technical work of the cycle to be equal to the supplied heat Qp, and it is also impossible to reach infinitely high temperatures or absolute zero, i.e., it is impossible to achieve efficiency η = 1 (J. GRUBER).
An example of a cyclic process is shown in the following figures.
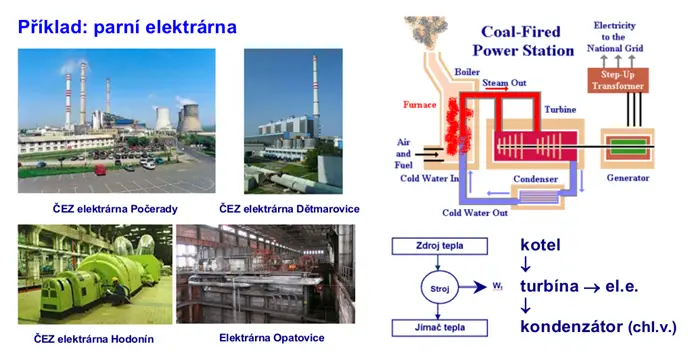
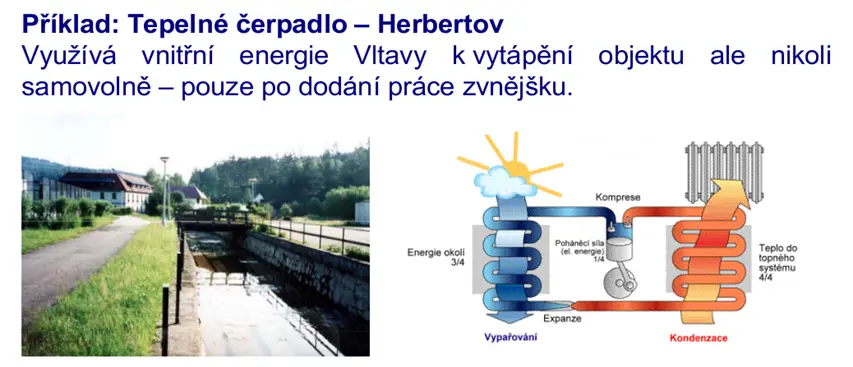
Natural and unnatural events, reversible and irreversible events
The first law of thermodynamics is known as the quantitative law because it allows for the occurrence of so-called unnatural phenomena (e.g., the spontaneous transfer of heat from a colder body to a warmer one). The most general definition of the second law of thermodynamics states: “Spontaneous processes in nature tend toward less ordered states” (J. GRUBER)8.
Reversible processes (reversible) can be returned to the initial state, passing through various states back to the initial state; reversible processes are also equilibrium (quasi-static), meaning the observed states are stable (in terms of temperature, physical and chemical composition, forces acting, etc., the thermodynamic system has the same physical and chemical properties at all points). The second law of thermodynamics denies the possibility of reversible spontaneous processes.
Irreversible processes are non-equilibrium and do not return to the original state.
It happens at a constant thermodynamic quantity
Processes with constant thermodynamic quantities are as follows, the process (for simplicity, assuming an ideal gas) according to the source 9:
Isothermal – a process in which there is no change in temperature (dT=0; dU=CVdT=0); all the heat supplied is used to perform work (dQ=dW).
Isochoric – a process in which there is no change in volume (dV = 0); all the heat supplied is used to increase the internal energy (dQ = dU).
Isobaric – a process in which there is no change in pressure (dp=0); the heat absorbed by an ideal gas in an isobaric process equals the sum of the increase in its internal energy and the work done by the gas (dQ=dU+W; dW=pdV).
Adiabatic – no heat exchange takes place between the gas and the surroundings (as in isolated systems; dQ=0), or the process occurs so quickly that no heat exchange happens – real processes are often on the border between isothermal and adiabatic (i.e., polytropic process); entropy does not change in an adiabatic process; the system performs work at the expense of its internal energy (dW=-dU); during adiabatic compression of gas in a container, the gas temperature and internal energy increase as work is done by external force on the piston; during adiabatic expansion, the gas performs work, and its temperature and internal energy decrease; adiabatic expansion is used to achieve low temperatures; adiabatic compression is used in diesel engines – the air temperature is raised to the ignition temperature of diesel by adiabatic compression.
Isoentropic – a process in which there is no change in entropy (dS=0).
In practice, it is useful to assess the feasibility of spontaneous processes, which can be derived from the second law of thermodynamics, as shown in the following image.
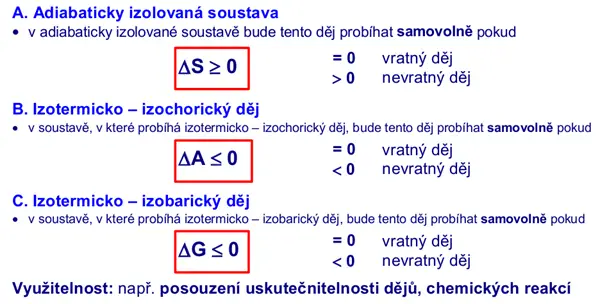
Exothermic and endothermic processes, thermochemistry
Exothermic process is a thermal reaction in which the thermodynamic system releases heat, i.e., the change in enthalpy is negative dH < 0.
Endothermic process is a thermal reaction in which the thermodynamic system absorbs heat, i.e., the change in enthalpy is positive dH > 0.
Exothermic and endothermic processes are addressed by chemical thermodynamics.
Chemical thermodynamics, i.e., thermochemistry, applies the basic principles of thermodynamics in systems where physical processes (e.g., phase transitions, i.e., changes) occur, physicochemical processes (e.g., dissolution), and chemical processes (e.g., chemical reactions). These physical, physicochemical, and chemical processes are always associated with a thermal reaction.
Phase transition – “is a sudden change in the macroscopic properties of the thermodynamic system (phase) when a thermodynamic variable (e.g., temperature) changes. During a phase transition, some macroscopic property of the substance, such as density, thermal conductivity, specific heat capacity, etc., always changes suddenly. The transition between phases is usually associated with a certain latent heat, i.e., the energy that the substance must absorb or release for the phase transition to occur.”11. That is, a thermal reaction. It is not a chemical reaction.
First-order phase transition – the change in the state of matter of the thermodynamic system (i.e., freezing or crystallization, melting, evaporation or condensation, sublimation or desublimation); it depends on temperature and pressure [5],
Second-order phase transition – the emergence of a ferromagnetic phase and piezoelectric properties in materials at the Curie point (i.e., the temperature at which the substance loses its ferromagnetic or piezoelectric properties) and the onset of superconductivity in metals and certain other substances at low temperatures T12.
Physicochemical processes can be associated with the dissolution or dilution of certain substances, so thermochemistry monitors the so-called heat of dissolution (ΔHROZP) and heat of dilution (ΔHZŘEĎOVACÍ).
Heat of dissolution – the heat that the system exchanges with the surroundings when a substance dissolves at constant pressure and temperature, i.e., an isothermal – isobaric process:
– for most substances, ΔHROZP > 0, i.e., the system absorbs heat, which lowers the temperature of the surroundings; the heat is used as energy to disrupt the crystal lattice and release the particles,
– in some cases (e.g., dissolution of NaOH in water) ∆HROZP < 0, i.e., the system releases heat, which increases the temperature of the surroundings; this is called ion solvation (the splitting of the dissolved substance molecule by solvent molecules).
Heat of dilution is the heat that the system exchanges with the surroundings when diluting a solution from concentration c1 to concentration c2, also an isothermal – isobaric process. An example of heat of dilution is the strong exothermic reaction during acid dilution: “Always add acid to water (solution), but never the other way around”13.
For chemical reactions, we will limit ourselves to the basic thermochemical laws that also apply to the above thermochemical processes, see (J. BRÍŽĎALA) 14:
1. First thermochemical law – “The value of the reaction heat for the direct and reverse reaction is the same, except for the sign.”
2. Second thermochemical law – “The resulting reaction heat is dependent only on the initial and final state of the reaction. The transient states of the chemical reaction do not affect it.”.
Safety importance
Thermodynamics is present in almost all technical fields, which is why it is very important. Understanding the basic principles of thermodynamics is necessary for recognizing the risks of technical systems, primarily from the safety perspective.
Basic physical principles must also be considered in the abstract world, which is increasingly emphasized within the context of digitization and Industry 4.0, see our previous article. In the realm of abstraction and cyber systems, it is necessary to perceive reality because cyber-abstract elements are linked with physical ones (cyber-physical systems). Poor regulation caused by a faulty or low-quality software design can lead to extreme thermodynamic phenomena and disasters.
In the next part about thermodynamics, we will focus on extreme phenomena occurring in storage tanks, such as cisterns or, for example, in nuclear power plants within the steam cycle of nuclear reactors.